Mitochondria health the role of electrotherapy | NuroKor Evidence
Contents
Introduction
Mitochondria Anatomy and Function
Mitochondrial Damage and Dysfunction
Oxidative Stress Induced Mitochondrial Damage and Dysfunction
Medication Induced Mitochondrial Damage and Dysfunction
Electrotherapy Improves Mitochondrial Function
Electrotherapy is an Effective Pain Treatment & Helps Reduce Analgesics Use
Combating Mitochondria Dysfunction
Mitochondria Health The Role Of Electrotherapy
Introduction
This article provides you with important information about the mitochondria, which are organelles present in the cells, and are crucial for the production of energy and other functions.
Mitochondrial dysfunction is a condition that can result from genetic defects, or can be caused by certain factors such as high levels of toxins and medications. This condition leads to impaired ability to produce energy, and to the development of a number of related diseases.
Currently there is not a single treatment to resolve mitochondrial dysfunction, but there are different interventions that can help prevent and treat this condition. These include electrotherapy, a balanced diet and physical activity. Electrotherapy can help in different ways, including treating pain and thus avoid the side effects of drugs, stimulate the muscle in a way to mimic physical exercise, and provide the energy to help cells function and repair.
Mitochondria Anatomy and Function
Mitochondria are rod-shaped structures and are extremely important organelles found in the cytoplasm of the cells. Mitochondria are composed of compartments that carry out specialised functions, and include the outer membrane, the inter membrane space (the space between the two membranes), the inner membrane, and the matrix. The outer membrane covers the entire surface of these organelles, while the inner membrane has many folds called cristae, which increase the surface area of the membrane, while the matrix is the space inside the inner membrane.
Because of their central role in energy production, mitochondria are often referred as the powerhouse of the cells.
Mitochondria consume about 85% of the oxygen utilised by the cell during ATP production. Mitochondria are the site of aerobic respiration, which is a complex energy pathways, and includes the Krebs cycle and oxidative phosphorylation, and each of these occurs in a specific region of the mitochondria. The Krebs cycle takes place in the matrix of the mitochondria, while oxidative phosphorylation takes place in the inner mitochondrial membrane. In turn, oxidative phosphorylation comprises two components: the electron transport chain and chemiosmosis, respectively.
During these metabolic processes the substrate is broken-down and the energy released is utilised for adenosine triphosphate (ATP) synthesis. The potential energy within the ATP molecule is then harnessed to power the physiological processes required to sustain life. Consequently, ATP is considered the energy currency of all living organisms.
It is estimated that a human on average requires 65 kg of ATP per day, and mitochondria, represent the main producers of cellular ATP. In essence, ATP is constantly hydrolysed (broken-down by adding a water molecule) into adenosine diphosphate (ADP) and phosphate (Pi) and free energy is released, while, the energy extracted from food is used to resynthesize ATP.
Mitochondria are responsible for producing about 90% of the cellular energy.
In addition to aerobic respiration, mitochondria are involved in a number of other critically important physiological processes, such as the synthesis of proteins and amino acid, nucleotide and fatty-acid metabolism, as well as lipid, quinones and steroid biosynthesis, calcium signalling, iron-sulfur (Fe/S) cluster biogenesis, apoptosis, and ion homeostasis.
Mitochondria are present in all human cells except mature erythrocytes. Cellular energy requirements control the number of mitochondria present in each cell. A single somatic cell can contain from 200 to 2000 mitochondria, which depends on the type of cell and its metabolic activity. Skeletal and cardiac muscles, liver, brain and kidneys represent the most metabolically active tissues, and contain the largest number of mitochondria.
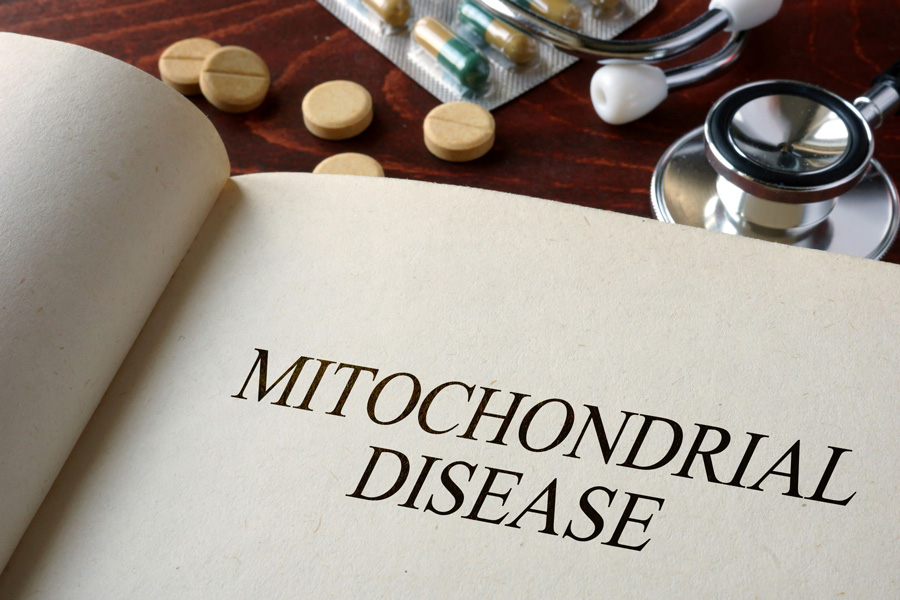
Mitochondrial Damage and Dysfunction
The integrity of mitochondrial function is fundamental to the cell life. Mitochondrial dysfunction leads to disruption of cell function, development of disease or even death. The advance of physiology and medicine has helped elucidating the role that the mitochondria play in health and performance.
In fact, since mitochondria are involved in numerous vital physiological processes, damage to these cell organelles leads to the deterioration of many bodily functions and to the pathogenesis of a wide range of seemingly unrelated disorders.
The number of diseases resulting from mitochondria damage and dysfunction is really vast, and include:
- Chronic fatigue syndrome
- Diabetes
- Cardiomyopathy
- Bipolar disease
- Transient ischemic attack
- Epilepsy
- Coronary artery disease
- Sarcopenia
- Stroke
- Neuropathic pain
- Dementia
- Fibromyalgia
- Alzheimer's disease
- Retinitis pigmentosa
- Parkinson's disease
- Poor growth
- Ataxia
- Primary biliary cirrhosis
- Schizophrenia
- Anxiety disorders
- Cancer
- Exercise intolerance
Luft and colleagues first described mitochondrial disease in 1962, when a young woman presented with myopathy (a disease of muscle tissue), polydipsia (abnormally great thirst) with polyuria (excessive production of diluted urine) heat intolerance and hypermetabolism, despite normal thyroid function.
The root cause was an uncoupling of oxidative phosphorylation that is the major cellular energy-producing pathway. In essence, uncoupling of oxidative phosphorylation leads to hit production without generating ATP.
Since this first documented case, other studies have shown that mitochondrial dysfunction is involved in many pathologic and toxicologic conditions.
The symptoms mitochondrial dysfunction, the rate of progression and age of onset vary across individuals, which makes it difficult to diagnose at an early stage. Initially the symptoms can be mild and may not resemble any known mitochondrial disease. Besides, symptoms such as muscle pain, fatigue, shortness of breath, and abdominal pain can easily be mistaken for fibromyalgia, chronic fatigue syndrome, collagen vascular disease, or psychosomatic illness. Mitochondrial dysfunction can result from a genetic defect or an inherited condition, but also can be caused by other factors such as high levels of oxidative stress or certain types of medications.
Oxidative Stress Induced Mitochondrial Damage and Dysfunction
Damage to the mitochondria can be caused by free radicals and the related reactive oxygen species, which include any molecule that contains one or more unpaired electrons.
Free radicals can be endogenous and are products of many metabolic pathways. For instance, free radicals are generated by the mitochondria themselves during cellular respiration. Some free radical exist in a controlled form and are part of normal functions, while others exist in a free form and interact with various tissue components. These interactions can cause tissue injury and diseases, including damage to the mitochondria.
Free radicals can be neutralised by the body’s antioxidant system, which includes the action of specific enzymes, vitamins, minerals, amino acids and other bioactive compounds. The cooperative action of all these nutrients and bioactive compounds helps prevent the potential negative effects of free radicals.
However, when the amount of free radicals produced is greater that the antioxidant system ability to neutralise them, oxidative damage occurs as a result.
Because the mitochondria produce considerable amount of free radicals during cellular respiration, consequently they are particularly at risk of oxidative damage, which can accumulate within the mitochondrial structure. The mitochondria elements that are especially vulnerable to free radicals include mitochondrial DNA, proteins, lipids, and enzymes.
Such damage leads to decreased mitochondrial function, and also increases the cellular requirements for energy repair processes, compromising further their function and compounding the problem. In essence, mitochondrial dysfunction can result in a feedforward mechanism, whereby mitochondrial damage causes additional damage.
Medication Induced Mitochondrial Damage and Dysfunction
There is sufficient evidence suggesting that medication can damage mitochondria, both directly and indirectly.
The direct damage caused by medication include inhibition of mitochondrial DNA transcription of the electron transport chain complexes and damage to their components, and inhibition of key enzymes necessary for glycolysis and beta oxidation.
While the indirect damage caused by medication includes, increased free radicals production, decreased endogenous antioxidant, and depletions of essential nutrients required for the synthesis and proper function of mitochondrial enzymes and electron transport chain complexes.
Mitochondrial damage can explain many of the numerous side effects resulting from different types of medications.
Electrotherapy Improves Mitochondrial Function
Currently, there is no single treatment available to resolve mitochondrial dysfunction, and the first and best line of defence remains prevention.
This is most likely to be achieved through a comprehensive, integrated multidisciplinary approach that includes various therapeutic components. Such approach may include reducing or eliminating the medications that induce toxicity, reduce oxidative stress, increase physical exercise and increase antioxidant intake through a balanced diet.
Electrotherapy can help prevent mitochondrial damage and improve mitochondrial function.
Electrotherapy is an approach that utilises electrical impulses, generated by specifically designed devices and delivered through electrodes placed on the skin.
Electrotherapy comprises different modalities, including peripheral nerve stimulation (PNS) also known as transcutaneous electrical nerve stimulation (TENS), neuromuscular electrical stimulation (NMES), and microcurrent stimulation (MCS). Each of these modalities delivers electrical impulses in a specific way and thereby achieving therapeutic, wellbeing and fitness related benefits.
The concept of utilising formulations developed in all 3 key areas of electrotherapy PNS, NMES and MCS, respectively, was a primary consideration in the development of NuroKor’s KorOS5 app system. This multimodal electrotherapy approach has the potential of bringing about a wider range of therapeutic and wellbeing related benefits.
Physical exercise is a powerful stimulus that improves mitochondria function, as well as cellular and whole body health.
However, physical exercise adherence is often transient and/or partial, and there is evidence suggesting that a large percentage of individuals remain sedentary. Furthermore, health conditions and obesity in many cases represent barriers to exercise participation.
NMES application elicits involuntary muscle contractions, and has been shown to bring about both health and fitness related benefits, including improving mitochondrial function.
These benefits are similar to those resulting from voluntary contractions occurring during physical exercise. Mitochondria are dynamic organelles with the ability to fuse and divide (fission), and in this process are involved various proteins. This process is important for maintenance of functional mitochondria, and is termed mitochondrial dynamics.
A study carried out by Kitaoka and colleagues demonstrated that a specific protocol formulation of NMES applied in mammals skeletal muscles to mimic acute and chronic resistance exercise, increases the production of proteins involved in mitochondrial fusion and fission, and this may contribute to improve mitochondrial and muscle function.
Besides, a previous study conducted in Japan showed that NMES administration in mammal skeletal muscles activates a signalling compound associated with augmented muscle protein synthesis, which in the long-term induces significant increases in muscle size and strength.
A study conducted by Erickson and colleagues investigated the effect of NMES on skeletal muscle oxidative capacity. The study included individuals with motor-complete spinal cord injury, and the initial NMES session took place in the laboratory under the supervision of trained research personnel.
Participants were given instructions and guidance how to use the devices, and subsequently completed home-based endurance NMES training. NMES was applied on the quadriceps muscles of both legs, and frequency and duration of the sessions were increased progressively over the course of 16 weeks based on the onset of muscle fatigue. While, the NMES intensity varied among participants and was selected based on visual observation of vigorous muscle contraction, and remained constant throughout the training.
The results demonstrated that skeletal muscle oxidative capacity increased significantly after 16 weeks of home-based endurance NMES training. Because the NMES training varied among participants, consequently, the skeletal muscle oxidative capacity improvements ranged from 14% to 387%. This wide range in responses probably reflect the intensity and volume of the electrical stimulus applied to the muscles.
A previous study has shown that muscle endurance is improved after 18 weeks of NMES administration.
Since muscle oxidative capacity and muscle endurance are heavily dependent on mitochondrial efficiency, therefore, the improvements of these variables observed after NMES administration imply an improvement in mitochondrial function.
Furthermore, Rostrup and colleagues found that electrical and thermal stimulation combined, and administered to obese individuals for 8 weeks increased both lipolytic activity and mitochondrial activity in abdominal adipose tissue. The results also showed a significant improvement in functional capacity towards a higher fatigue resistance.
In addition, a case study of a 39-year-old man with a motor complete spinal cord injury, underwent 24 weeks of endurance NMES training. The findings showed that NMES endurance training, in individuals with motor complete spinal cord injury, can increase mitochondrial capacity to comparable levels measured in non-paralyzed muscles of sedentary able-bodied controls.
Other studies also demonstrated that electrotherapy has positive effects on mitochondria health. Mitochondrial membrane potential (the potential difference between ions inside and outside the membrane), is required for mitochondrial oxidative phosphorylation and proton transmembrane transport, and reflects mitochondrial function.
The decrease of mitochondrial membrane potential is the precursor of cell oxidative damage. For instance, in myocardial aging cells are observed a reduction in mitochondrial membrane potential, increased proton leakage and augmented free radicals production.
Skeletal muscle health is dependent on the optimal function of its mitochondria. Increased skeletal muscle tissue damage is related to changes in mitochondrial membrane potential, due to elevated free radicals level and DNA oxidative damage. Any change in skeletal muscles mitochondrial function affects muscle health and performance. Studies have shown that physical exercise improves skeletal muscle mitochondrial health, and is also beneficial to maintain the mitochondrial membrane potential of aging mammalian myocardial cells.
Similarly, a study conducted in China found that 60 and 120 minutes of electrotherapy administration promoted mitochondrial membrane potential recovery, which is important for maintaining mitochondrial health.
Furthermore, there is evidence suggesting that MCS application in mammalian tissue, increases ATP concentrations, re-establishes the nerve cell membrane potential to healthy levels, and reduces intracellular metabolic waste. This also allows new metabolites to enter the exhausted cell, and thus facilitating the regenerative functions to be re-established. Also, MCS administration stimulates the amino acid transport across the cell membrane.
The effects of MCS on both amino acid transport and ATP production, contribute to increasing protein synthesis activity. All these positive effects are important for cellular function.
Electrotherapy is an Effective Pain Treatment & Helps Reduce Analgesics Use
Analgesics and inti-inflammatory medications are frequently over prescribed and overused, but often they have numerous and serious side effects, including causing mitochondrial damage and dysfunction.
Electrotherapy represents a viable and safe intervention to eliminate or reduce the use of such drugs.
Indeed, PNS and MCS have been applied in a variety of contexts, and numerous studies demonstrated both in laboratory and clinical settings that they are effective in treating numerous conditions including cancer bone pain, chronic hip arthritis pain, neck and back pain, chronic pelvic floor pain, as well as acute, chronic and trauma and post-operative pain. In many instances the administration of these electrotherapeutic modalities alone resulted in pain relief, while in other cases they reduced significantly the need for analgesics.
Moreover, electrotherapy application reduces inflammation and oedema, and improves wound healing and tissue repair.
These beneficial effects are extremely important also because the elimination or reduction of analgesics, inti-inflammatory and other drugs use helps prevent mitochondrial dysfunction and other medications related side effects.
Collectively these studies show that electrotherapy represents an efficacious technique that promotes mitochondrial function and health.
Combating Mitochondria Dysfunction
Despite the enormous investment by the NHS and other health organisations, the frequency of mitochondrial dysfunction related diseases is increasing dramatically, impacting patients, families and societies. Diseases caused by mitochondria dysfunction can be varied, affect any organ or tissue and can appear anytime throughout life.
Furthermore, cells and bodily systems with higher level of metabolic activity, such as the brain, heart, skeletal muscles, kidneys and the endocrine system, appear to be most vulnerable to mitochondrial dysfunction. The diseases affecting such organs are inherently complex, and the severity of the symptoms varies among individuals.
Therefore, all these factors need to be taken into consideration when formulating the interventions, and also a shift in paradigm it is likely to be required.
Such shift could involve departing from the traditional “pharmacology treats all” approach, and rather seek to implement an approach that includes the most appropriate treatments in order to maximise clinical outcomes, and minimise side effects. Such approach could be implemented for preventing and treating mitochondrial dysfunction, which would help eradicate a number of devastating diseases that affect an increasing number of people.
The evidence provided by a numerous of studies suggests that electrotherapy has an important role to play in promoting mitochondrial function and health.
Electrotherapy has various advantages, as this technique is non-invasive, non-pharmacological, non-toxic, non-addictive and safe. Also, electrotherapy application is virtually side effects free and cost effective, especially in the long term.
Furthermore, the electrotherapy devices are small, light and portable, and studies have shown that after a minimum of instructions and guidance, patients are able to self-administer electrotherapy effectively, from the comfort of their home as needed. Importantly, the patients are able to adjust the electrical stimulation intensity, as well as the duration and frequency of the therapeutic sessions according to their necessities, rendering this technique a more personalised treatment.
Thus, it would be highly desirable to incorporate electrotherapy within the standard care routine, in order to prevent and treat mitochondrial dysfunction.
It should be noted that electrotherapy in itself will not replicate the outcomes of the studies noted in this article. The protocols utilised within the test parameters would need to be studied and applied as part of any treatment formulation with the potential to reproduce results.
At NuroKor, we value the efficacy, safety and versatility of electrotherapy, which delivers a flow of pure energy that activates and fuels the body’s elaborate healing mechanisms. We design handheld ultra-wearable, multimodal personal electrotherapy devices based on medical evidence, combined with cutting-edge technology, to help treat diseases, manage pain and promote health and wellbeing.
About NuroKor:
Founded in 2018, NuroKor is a company committed to the development of bioelectronic technologies. NuroKor develops and formulates programmable bioelectronic software for clinical and therapeutic applications, in a range of easy to use, wearable devices. It provides the highest-quality products, delivering personalised pain relief and recovery support and rehabilitation to patients.
References:
Atteia, A., Adrait, A., Brugie` re, S., Tardif, M., van Lis, R., Deusch, O., Dagan, T., Kuhn, L., Gontero, B., Martin, W., et al. (2009). A proteomic survey of Chlamydomonas reinhardtii mitochondria sheds new light on the metabolic plasticity of the organelle and on the nature of the alphaproteobacterial mitochondrial ancestor. Mol. Biol. Evol. 26, 1533–1548.
Aw, T. Y., Jones, D. P., Nutrient supply and mitochondrial function. Annu. Rev. Nutr. 1989, 9, 229–251.
Berg H. Electrostimulation of cell metabolism by low frequency electric and electromagnetic fields. Biochemistry and Bioenergetics 1993. 38: 153-159
Bishop, D.J., Granata, C., and Eynon, N. (2014). Can we optimise the exercise training prescription to maximise improvements in mitochondria function and content? Biochim Biophys Acta 1840, 1266-1275.
Blanc M. NA, K and ATPase function in alternating electric fields. 1992. FASEB Journal. 1992. 6:2434-2438
Bolognani L. Majni G. Costato M., and Milani M. ATPase and ATPsynthase activity in myosin exposed to loer laser and pulsed electromagnetic fields. Bioelectrochemistry and Bioenergetics. 1993. 32: 155-164
Brookes, P. S., Yoon, Y., Robotham, J. L., Anders, M. W., Sheu, S. S., Calcium, ATP, and ROS: A mitochondrial lovehate triangle. Am. J. Physiol. Cell Physiol. 2004, 287, C817–833.
Bua, E. A., McKiernan, S. H., Wanagat, J., McKenzie, D., Aiken, J. M., Mitochondrial abnormalities are more frequent in muscles undergoing sarcopenia. J. Appl. Physiol. 2002, 92 2617–2624.
Buist, R., Elevated xenobiotics, lactate and pyruvate in C. F.S. patients. J. Orthomol. Med. 1989, 4, 170–172.
Calvo, S.E., and Mootha, V.K. (2010). The mitochondrial proteome and human disease. Annu. Rev. Genomics Hum. Genet. 11, 25–44.
Carley and Wainapel. Electrotherapy for acceleration of wound healing: low intensity direct current. Archives of Physical Medicine and Rehabilitation. 1985. vol. 66.
Casey AG, McKernan MP and Reb J.H. Transcutaneous Electrical Nerve Stimulation (TENS) in the Emergency Department for Pain Relief: A Preliminary Study of Feasibility and Efficacy. Western Journal of Emergency Medicine. 2018. Volume 19, No. 5.
Chance, B., Sies, H., Boveris, A., Hydroperoxide metabolism in mammalian organs. Physiol. Rev. 1979, 59, 527 –605.
Cheng, N, Van Hoof H, Bockx E, Hoogmartens MJ, Mulier JC, De Dijcker FJ, Sansen WM, De Loecker W. The effects of electric currents on ATP generation, protein synthesis, and membrane transport of rat skin. Clinical Orthopaedics. 1982. 171:264-72.
Choi YD and Lee JH. Edema and pain reduction using transcutaneous electrical nerve stimulation treatment. J. Phys. Ther. Sci. 2016. 28: 3084–3087
Cogalniceanu G. Radu M. Fologea D. Moisoi N., and Brezeanu A. Stimulation of tobacco roots regeneration by altenating weak electric field. Bioelectrochemistry and Bioenergetics. 1998. 44 (2), 257-260
Cohen, B. H., Gold, D. R., Mitochondrial cytopathy in adults: What we know so far. Cleve. Clin. J. Med. 2001, 68, 625 – 626, 629–642.
Dong HL, WU HY, Zhao J.et al. Effect of Electrical Stimulation at Different Time on Mitochondrial Function of C2C12 Myotubes. J Biomol Res Ther. 2018, 7:2 DOI: 10.4172/2167-7956.1000162
Duchen, M. R., Mitochondria in health and disease: Perspectives on a new mitochondrial biology. Mol. Aspects Med. 2004, 25, 365–451.
Dudley GA, Abraham WM, Terjung RL. Influence of exercise intensity and duration on biochemical adaptations in skeletal muscle. Journal of applied physiology: respiratory, environmental and exercise physiology. 1982; 53(4):844–850.
Einat, H., Yuan, P., Manji, H. K., Increased anxiety-like behaviors and mitochondrial dysfunction in mice with targeted mutation of the Bcl-2 gene: Further support for the involvement of mitochondrial function in anxiety disorders. Behav. Brain. Res. 2005, 165, 172 –180.
Erickson ML, Terence MS, Ryan E. et al. Endurance neuromuscular electrical stimulation training improves skeletal muscle oxidative capacity in individuals with motor-complete spinal cord injury. Muscle Nerve. 2017. 55(5): 669–675. doi:10.1002/mus.25393.
Fulle, S., Mecocci, P., Fano, G., Vecchiet, I., et al., Specific oxidative alterations in vastus lateralis muscle of patients with the diagnosis of chronic fatigue syndrome. Free Radic. Biol. Med. 2000, 29, 1252 –1259.
Gawryluk, R.M.R., Chisholm, K.A., Pinto, D.M., and Gray, M.W. (2014). Compositional complexity of the mitochondrial proteome of a unicellular eukaryote (Acanthamoeba castellanii, supergroup Amoebozoa) rivals that of animals, fungi, and plants. J. Proteomics 109, 400–416.
Green, K., Brand, M. D., Murphy, M. P., Prevention of mitochondrial oxidative damage as a therapeutic strategy in diabetes. Diabetes 2004, 53, S110–118.
Gollnick PD. Metabolism of substrates: Energy substrate metabolism during exercise and as modified by training. Federation Proceedings. 1985. 44:353-56
Gollnick PD. Riedy M. Quintiskie JJ., and Bertocci LA. Differences in metabolic potential of skeletal muscle membrane and their significance for metabolic control. J. Exper. Biol. 1985. 115: 191-195
Gray, M. W., Origin and evolution of mitochondrial DNA. Annu. Rev. Cell Biol. 1989, 5, 25–50.
Jequier E, and Flatt J. Recent advances in human bioenergetics. News in physiological sciences. 1986. 1:112-14
Jin DM, Xu Y, Geng DF et al. Effect of transcutaneous electrical nerve stimulation on sympathetic peripheral neuropathy: a meta-anausis of randomized trails. Diabetes Res Clin Pract. 2010 Jul;89(1):10-5. doi: 10.1016/j.diabres.2010.03.021. Epub 2010 May 26.
Kehrer JP, and klots LO. Free radicals and the related reactive species as mediators of tissue injury and diseases: implication for health. Crit Rev Toxicol. 2015;45(9):765-98. doi: 10.3109/10408444.2015.1074159.
Kitaoka Y, Ogasawara R, Tamura Y, et al. Effect of electrical stimulation-induced resistance exercise on mitochondrial fission and fusion proteins in rat skeletal muscle. Appl. Physiol. Nutr. Metab. 40: 1137–1142 (2015) dx.doi.org/10.1139/apnm-2015-0184
Lee, B. Y. Al-waili N, Stubbs D, et al. (2009). "Ultra-low microcurrent in the management of diabetes mellitus, hypertension and chronic wounds: report of twelve cases and discussion of mechanism of action." Int J Med Sci 7(1): 29-35.
Lee, C.P., Taylor, N.L., and Millar, A.H. (2013). Recent advances in the composition and heterogeneity of the Arabidopsis mitochondrial proteome. Front. Plant Sci. 4, 4.
Liu, J., Killilea, D. W., Ames, B. N., Age-associated mitochondrial oxidative decay: Improvement of carnitine acetyltransferase substrate-binding affinity and activity in brain by feeding old rats acetyl-L-carnitine and/or R-alpha-lipoic acid. Proc. Natl. Acad. Sci. USA 2002, 99, 1876–1881.
Luft, R., Ikkos, D., Palmieri, G., Ernster, L., Afzelius, B., A case of severe hypermetabolism of nonthyroid origin with a defect in the maintenance of mitochondrial respiratory control: A correlated clinical, biochemical, and morphological study. J. Clin. Invest. 1962, 41, 1776 –1804.
Moraji M. Asai T., and Tatebe W. Primary root growth rate of Zea Mays seedlings grown in an alternating magnetic field of different frequencies. Bioelectrochemistry and Bioenergetics. 1998. 44 (2), 271-273
Odell RH, and Sorgnard RE. Anti-inflammatory Effects of Electronic Signal Treatment. Pain Physician 2008; 11:891-907 • ISSN 1533-3159
Ogasawara, R., Kobayashi, K., Tsutaki, A., Lee, K., Abe, T., Fujita, S., et al. mTOR signaling response to resistance exercise is altered by chronic resistance training and detraining in skeletal muscle. J. Appl. Physiol. 2013. 114: 934–940. doi:10.1152/japplphysiol.01161.2012.
Ogasawara, R., Sato, K., Matsutani, K. et al. The order of concurrent endurance and resistance exercise modifies mTOR signaling and protein synthesis in rat skeletal muscle. Am. J. Physiol. Endocrinol. Metab. 2014. 306: E1155–E1162. doi:10.1152/ajpendo.00647.2013. PMID:24691029.
Panigrahi, A.K., Ogata, Y., Zı´kova´ , A., Anupama, A., Dalley, R.A., Acestor, N., Myler, P.J., and Stuart, K.D. (2009). A comprehensive analysis of Trypanosoma brucei mitochondrial proteome. Proteomics 9, 434–450.
Park, J. H., Niermann, K. J., Olsen, N., Evidence for metabolic abnormalities in the muscles of patients with fibromyalgia. Curr. Rheumatol. Rep. 2000, 2, 131–140.
Platzer K. Obermeyer G., and Bentrup FW. AC fields of low frequency and amplitude stimulates tube polled growth possibly by stimulation of the plasma membrane proton pump. Bioelectrochemistry and Bioenergetics. 1997. 44 (1) 95-102
Ryan TE, Erickson ML, Young HJ, et al. Case Report: Endurance Electrical Stimulation Training Improves Skeletal Muscle Oxidative Capacity in Chronic Spinal Cord Injury. Arch Phys Med Rehabil 2013. 94 (12): 2559-2561
Rostrup E, Sletton G, Seidert R et al. Effect of combined thermal and electrical muscle stimulation on cardiorespiratory fitness and adipose tissue in obese individuals. Eur J Prev cardiol. 2014. 21 (10): 1292-9. doi: 10.1177/2047487313483606.
Russell AP, Foletta VC, Snow RJ, Wadley GD (2014) Skeletal muscle mitochondria: a major player in exercise, health and disease. Biochim Biophys Acta 1840: 1276-84.
Sabatier MJ, Stoner L, Mahoney ET, et al. Electrically stimulated resistance training in SCI individuals increases muscle fatigue resistance but not femoral artery size or blood flow. Spinal Cord. 2006; 44(4):227–233.
Savitha S, Panneerselvam C. Mitochondrial membrane damage during aging process in rat heart: potential efficacy of L-carnitine and DL alpha lipoic acid. Mech Ageing Dev 20016. 127: 349-55.
Searle RD, Bennet Mi, Johnson MI, et al. Transcutaneous Electrical Nerve Stimulation (TENS) for Cancer Bone Pain. J Pain Symptom Manage. 2009. 37(3):424-8. doi: 10.1016/j.jpainsymman
Sharma N, Rekha K, and Snirivasan J. Efficacy of transcutaneous electrical nerve stimulation in the treatment of chronic pelvic pain. J Midlife Health. 2017. 8(1): 36–39
Sharma H and Patel N. Effectiveness of TENS versus Intermittent Cervical Traction in patients with cervical radiculopathy. Int J Physiother Res. 2014. 2(6):787-92. ISSN 2321-1822 DOI: 10.16965/ijpr.2014.693
Shigenaga, M., Hagen, T., Ames, B., Oxidative damage and mitochondrial decay in aging. PNAS 1994, 91, 10771 –10778.
Spallone V, Lacerenza M, Rossi A, Sicuteri R, Marchettini P. Painful Diabetic Polyneuropathy: Approach to Diagnosis and Management. The Clinical journal of pain. Dec 30 2011.
Stavrovskaya, I. G., Kristal, B. S., The powerhouse takes control of the cell: Is the mitochondrial permeability transition a viable therapeutic target against neuronal dysfunction and death? Free Radic. Biol. Med. 2005, 38, 687–697.
Stork, C., Renshaw, P. F., Mitochondrial dysfunction in bipolar disorder: Evidence from magnetic resonance spectroscopy research. Mol. Psychiatry 2005, 10, 900–919.
Rich, P. Chemiosmotic coupling: The cost of living. Nature 2003, 421, 583.
Stanley WC, Connett RJ. Regulation of muscle carbohydrates metabolism during exercise. FASEB Journal. 1991. 5:2155-9.
Tanaka, M., Kovalenko, S. A., Gong, J. S., Borgeld, H. J., et al., Accumulation of deletions and point mutations in mitochondrial genome in degenerative diseases. Ann. N. Y. Acad. Sci. 1996, 786, 102–111.
Ud-Din S., and Bayat A. Electrical Stimulation and Cutaneous Wound Healing: A Review of Clinical Evidence. 2014. Healthcare. 2, 445-467; doi: 10.3390/healthcare2040445
van der Zwaard S, Brocherie F, Kom BLG, et al. J Appl Physiol (1985). 2018 Jun 1; 124(6):1403-1412. doi: 10.1152/japplphysiol.00946.2017. Epub 2018 Feb 8.
Veltri, K. L., Espiritu, M., Singh, G., Distinct genomic copy number in mitochondria of different mammalian organs. J. Cell. Physiol. 1990, 143, 160–164.
Wei, Y. H., Lu, C. Y., Lee, H. C., Pang, C. Y., Ma, Y. S., Oxidative damage and mutation to mitochondrial DNA and agedependent decline of mitochondrial respiratory function. Ann. N. Y. Acad. Sci. 1998, 854, 155–170.
Wallace, D. C., A mitochondrial paradigm of metabolic and degenerative diseases, aging, and cancer: A dawn for evolutionary medicine. Annu. Rev. Genet. 2005, 39, 359 –407.
Witt, H. T., Schlodder, E., and Graber, P.: Membrane-bound ATP synthesis generated by an external electrical field. FEBS Lett. 69:272, 1976.
Yuan X Derya E. Arkonac DE. Pen-hsiu Grace Chao PG., and Vunjak-Novakovic G. Electrical stimulation enhances cell migration and integrative repair in the meniscus. Sci. Rep. 2014. 4:3673
Yunus, M. B., Kalyan-Raman, U. P., Kalyan-Raman, K., Primary fibromyalgia syndrome and myofascial pain syndrome: Clinical features and muscle pathology. Arch. Phys. Med Rehabil. 1988, 69, 451–454.
Zoladz JA, Koziel A, Broniarek I, Woyda-Ploszczyca AM, Ogrodna K, et al.Effect of temperature on fatty acid metabolism in skeletal muscle mitochondria of untrained and endurance-trained rats. 201.7 PLoS One 12: e0189456.
Zrimec A. Jerman I., and Lahajnar G. Alternating electrical current stimulated ATP synthesis in Escherichia Coli. Cell. Mol. Biol. Lett. 2002. Vol.7. No.1